Nowadays, the increasing amount of heat and electricity consumed by houses comes from renewable energy sources. A special case of renewable-based systems are bioenergy villages, which can be defined as villages, municipalities, settlements, or communities that produce and use most of their energy from local bioenergy and other renewable energy sources [1]. Wood-fired stoves have been a crucial source of heating and cooking, especially for millions of households worldwide, especially in regions with limited access to modern energy sources. In March 2023, the Polish government implemented a new regulation in the construction law and act on spatial planning and development, which aimed to streamline the procedures for constructing new one-family houses. Typically, these newly built one-family houses are heated using traditional gas-fired boilers, heat pumps, or pellet-fired boilers. Wood-fired stoves can support each of these central heat sources. While some homeowners have implemented co-generation systems with alternative energy sources, it is more common for these stoves to function as standalone heating units, providing both warmth and hot water for the household. The increased trend in constructing smaller one-family houses and their reliance on biomass-fired boilers and stoves for heating reflects the appeal of a more sustainable and traditional approach to living. It also raises concerns about potential environmental impacts, particularly related to air quality and emissions from burning biomass as the primary fuel source and frequently, the mixture of biomass and plastic materials which interact with each other while pyrolyzing emitting unexpected components of flue gases as a result [2]. From the environmental point of view, residential wood combustion affects local air pollution with emissions of carbon monoxide (CO), hydrocarbons (HC), nitrogen oxides (NOx, mainly NO and NO2), sulfur oxides (mainly SO2), and particulate matter (PM) [3] and even heavy metals [4]. In addition, acid gases, such as hydrochloric acid, heavy metals, volatile organic compounds (VOCs), and polycyclic aromatic hydrocarbons (PAHs), including benzo-a-pirene can be formed and emitted into the atmosphere [5]. Moreover, wood-fired stoves are fuel-dependent, which, in turn, indirectly impact deforestation and the entire environment. Consequently, they need proper maintenance and cleaning and can pose safety risks, including the potential of accidental fires or burns.
However, the conventional use of wood-fired stoves is often associated with several challenges, both technical and environmental impact. As Johansson et al., 2004 [6] reported, the technical issues may be combined with inefficient combustion and inconsistent heat distribution and boiler type. Installing modern stoves and connecting the old-type wood boiler to a heat storage tank or charging small batches of wood significantly improves the emission performance of the central heating units. Szramowiat-Sala et al., 2019 [3] showed that the application of an accumulation layer in stoves reduces the emission of gaseous pollutants, like NOx, and SOx, but the concentrations of CO, PM and several PM-bound polycyclic aromatic hydrocarbons increased. This emission performance was fully combined with technical parameters of the combustion process, like fuel load or stove type and even combustion phase. These facts were also confirmed by other authors like Li et al., 2023 [7] and Czech et al., 2018 [8]. However, as Karlsson et al., 2020 [9] noticed, fireplaces can be used to regulate emotion, promote joy, and decrease stress on one hand. On the other hand, fireplaces can be a serious source of indoor pollution, escalating human exposure to hazardous chemical compounds if not well-maintained.
As the popularity of small houses continues to grow, it becomes essential to consider ways to enhance their energy efficiency and minimize their ecological footprint. In January 2022, the Eco-design regulation was implemented in the European Union, which defines the cleanliness and effectiveness of domestic heating units fuelled by wood and other solid fuels. The Eco-design also limits the emission of pollutants up to 1500, 40, 2000, and 120 mg/m3 for CO, PM, NOx, and organic gas compounds (OGC), respectively [10]. The significance of discovering sustainable and cleaner solutions for wood-fired stoves cannot be overstated, given their widespread usage worldwide. While the stoves have served as a traditional and essential source of heating and cooking for generations, their conventional design and operation present significant environmental and health challenges. Addressing the environmental and energy efficiency challenges requires transitioning towards sustainable and cleaner solutions for wood-fired stoves. By adopting innovative technologies and design improvements, it is possible to enhance the energy efficiency of the stoves, leading to reduced fuel consumption and fewer greenhouse gas emissions. More efficient combustion can also significantly decrease harmful pollutants released into the atmosphere, thereby improving air quality and mitigating the impact on public health.
Several approaches can be explored to achieve these goals. Integration of modern combustion techniques, better insulation materials, and optimized air intake controls can enhance combustion efficiency and heat retention. Additionally, using cleaner and renewable biomass resources, such as wood pellets or briquet, can reduce emissions and promote sustainable practices [5]. Furthermore, according to the investigation presented by Juan and Sy, 2021 by integrating systems fuelled with biomass co-firing the reduction in coal use and greenhouse gas emissions may be achieved [11]. This could be combined with production of bioenergy from lignocellulose biomass conversion (i.e. units based on biomass, biogas or biooil combustion) [12]. Above mentioned technological solutions may be successively integrated with solar-powered systems (such as photovoltaic, concentrated solar power, etc.) leading to increase in the efficiency and renewability of the heat and power generation [13]. Solar-powered systems, as proved by Dehghan and Pfeiffer, 2017, can be an efficient solution of heat generation [14]. A huge progress has been made in automatization and optimization of processes of energy production, and energy efficiency elevation [15]. Above mentioned solutions serves the sustainability and must be developed respecting the sustainability rules at the same time, i.e. taking into account the questions of water scarcity and water saving during energy production [16]. Optimisation of heat distribution in building was the subject of investigations presented by Serra and Filho, 2019 [17]. Srbinovska and Cudneva-Blajer, 2019 examined the usage of energy by wireless sensor networks applied in domestic heating generators and environmental monitoring systems to extend their lifetime and durability [18]. Predictive models found their application in thermal assessment of buildings as it was showed by Steindl et al., 2019 [19]. Khan, 2020 developed intelligent real time algorithms in order to minimize the energy payment for each residential user, as well as reduce the peak to average ratio to overcome the drawbacks in the stability of electrical grid [20]. Forecasting of energy efficiency is also an area where artificial intelligence can be applied what was proved by Timur et al., 2020 [21]. An hourly linear optimisation model, in turn, was applied for the estimation of renewable energy supply in a residential area [22]. Similarly to Parrado-Hernando et al., 2024 on the base of several long-term scenarios presented the variability of renewable energy sources [23]. An energy characteristic study of a building in the aspect of CO2 generation was performed by Pireci and Vušanović, 2022 who presented different approaches in modelling and optimisation of energy efficiency [24].
However, exploration of innovative technologies focused on micro-cogeneration systems, thermoelectricity, Stirling engines or Rankine/Organic Rankine Cycle or other advanced ways of waste heat recovery applied also in an industry [25] may hold the key to improving the performance of wood-fired stoves and to alternatively re-use the heat generated by a stove converting it to electricity [26]. Thermoelectric generators (TEGs) offer a well-suited solution for harnessing energy from low-grade waste heat, making them compatible with various heating devices like boilers and stoves [27]. Despite their relatively low conversion efficiency, typically around 5%, TEGs boast several advantages, including direct heat-to-electrical energy conversion, lightweight design, silent operation, and the absence of mechanical vibrations. The use of TEGs to recover waste heat is particularly advantageous because the low conversion efficiency becomes less critical when the thermal energy input is essentially cost-free [28]. In the context of micro-cogeneration systems (μCHP) that incorporate a wood-fired stove and a thermoelectric generator, additional components are essential for seamless operation. These systems require regulators, battery charge controllers, batteries, and stove controllers to manage the power generation process effectively. The generated electrical power finds consumption in various components, such as an air throttle servo motor, controller, and cooling system. Furthermore, any surplus power can be utilized by household appliances, with the direct current (DC) power being converted to alternating current (AC) power through an inverter. Moreover, the presence of an accumulation layer in a stove is also a key factor. As defined, an accumulation layer in wood-fired stoves serves as a crucial component that improves the combustion process by storing excess heat and gradually releasing it to the indoor environment, ensuring more efficient heating [29] and indirect reduction of CO emission by improving the efficiency of fuel combustion [30].
By integrating TEGs into micro-cogeneration systems based on wood-fired stoves, a viable and efficient means of recovering waste heat is achieved, offering a sustainable approach to meeting electricity demands while utilizing the available thermal energy efficiently [31]. Such setups present promising prospects for advancing energy efficiency and reducing dependency on traditional power sources in various applications.
Finding sustainable and cleaner solutions for wood-fired stoves is crucial not only for mitigating environmental impacts and safeguarding public health but also for promoting energy security and sustainable development. Here, we propose an integration of wood-fired stoves with TEGs to improve the energy efficiency of manually operated heating devices and reuse the wasted heat generated by the stove walls and the high temperature inside the combustion chamber. In the paper, the most favorable conditions of fuel burning in a manually controlled stove to mount the TEGs were investigated.
The investigations discussed in this paper have been conducted using the specially developed experimental rig and experimental procedure. This section presents the most important facts connected with the materials and methods used during the tests.
The experimental rig was equipped with several components. The most important elements are listed below:
a wood-fired steel stove (with a heating capacity ranging from 8 to 16 kWth),
thermoelectric generators (a 45 We air-cooled TEG and a 100 We water-cooled TEG, designed for mounting on a flat hot surface),
the MRU ECO 3000 Plus analyzer of carbon monoxide (CO), carbon dioxide (CO2), oxide (O2), sulfur dioxide (SO2), and nitrogen oxides (NOx) in flue gas,
a dust probe for PM sampling,
measurement and control system with WAGO PFC200 PLC controller,
PC with CoDeSys software,
infrared camera NEC Thermo Tracer H2640 (features a 640 × 480 pixels image resolution, temperature range from 0 to 500 °C, sensitivity 0.06 °C, and measurement accuracy ±2°C/±2%).
A wood-fired steel stove used as a heat source is a unit designed for burning seasonal hardwood (with humidity up to 20%), and brown coal briquettes was used. The main component of the stove under consideration is the combustion chamber. The primary air is delivered through the gap located under the door, while the secondary air is delivered through the gap located above the door. The amount of primary and secondary air is controlled using one dedicated air throttle. The typical construction of the stove consists of fire clay, which raises the temperature in the combustion chamber to achieve better combustion. However, to enable the installation of the thermoelectric generator, the fire clay has been removed from the rear part of the combustion chamber (see Figure 1).
The view of the furnace with complete fire clay layer (a) and partially removed fire clay layer (b)
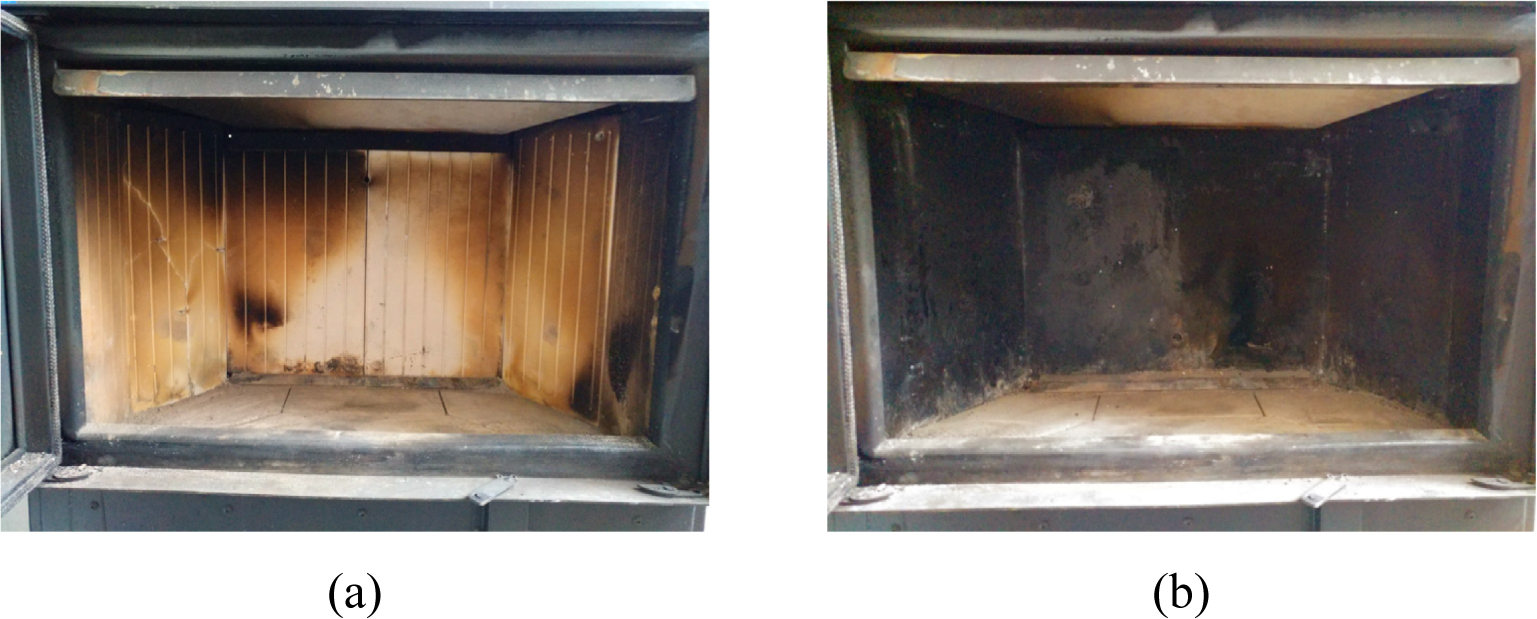
Thermoelectric generators have been mounted on the rear wall of the stove in the area where the fire clay layer had been removed. The air-cooled generator (TEG1) comes with two self-powered fans to dissipate the heat from the cold side and is designed to operate with a hot plate temperature of 350 °C. The second TEG (TEG2) is a water-cooling type with a matched output power of 100 W. The main parameters of the tested TEGs are presented in Table 1.
The main parameters of the tested thermoelectric generators
Parameter |
TEG1 |
TEG2 |
---|---|---|
Matched output power [W] |
45 |
100 |
Matched load output voltage [V] |
12 |
24 |
Matched load output current [A] |
3.8 |
4.2 |
Hot side temperature [°C] |
350 |
270 |
Cold side temperature [°C] |
30 |
30 |
Number of TE modules [-] |
6 |
8 |
Dimension size [mm] |
330 × 220 × 108 |
600 × 146 × 57 |
Weight [kg] |
8.0 |
7.8 |
Due to the temperature sensor location of the mounting bracket for the temperature sensor (placed in the central bottom part of the back wall of the stove), TEG2 was placed on the left side of the back wall (see Figure 2).
The view of the stove with a thermoelectric generator 1 (a) and a thermoelectric generator 2 (b) mounted on its rear wall [24]
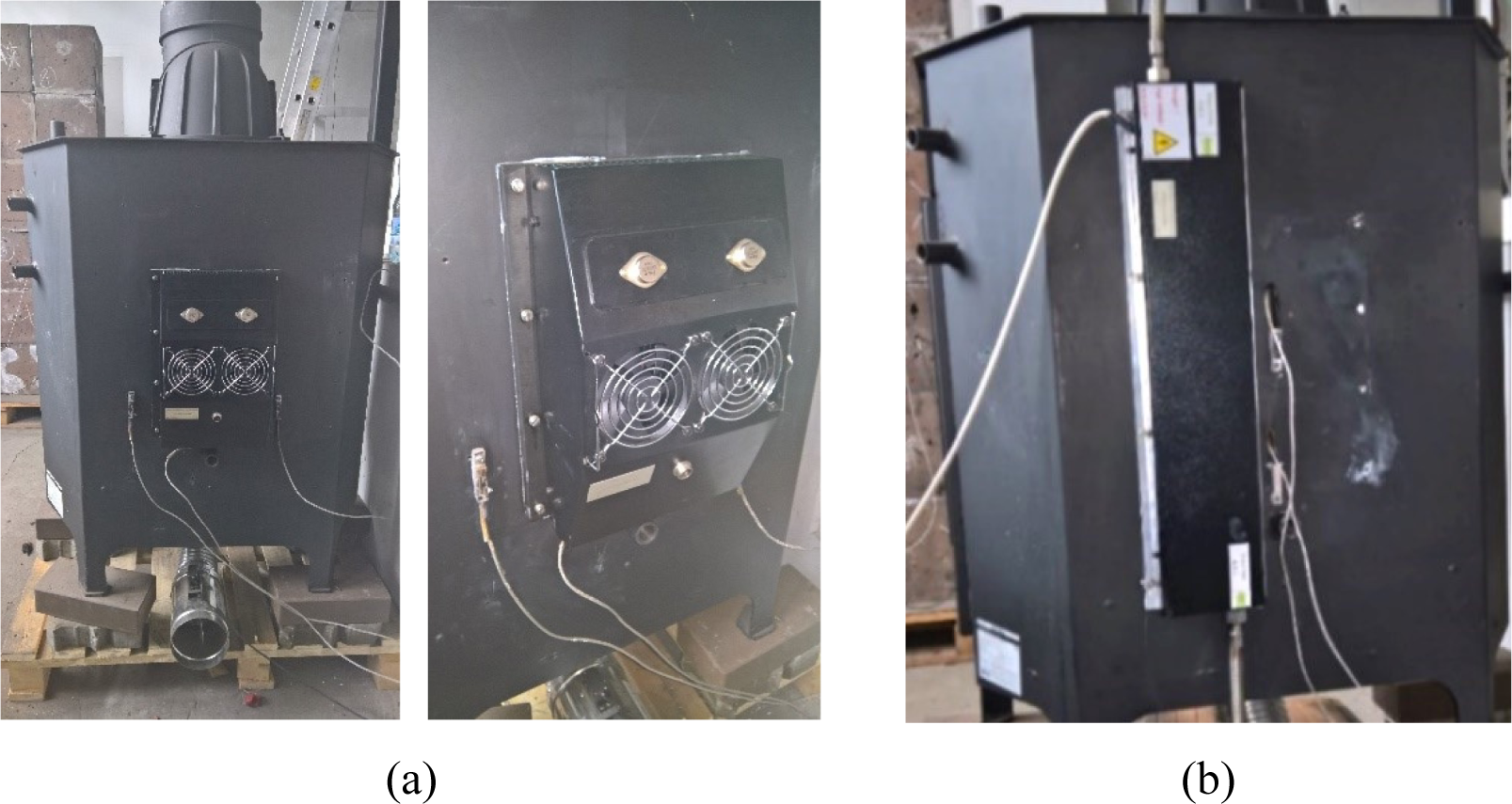
To assess the feasibility and effectiveness of TEGs integration with a manually operated stove Scenarios I-IV have been planned as presented in Figure 3.
The plan of measurements
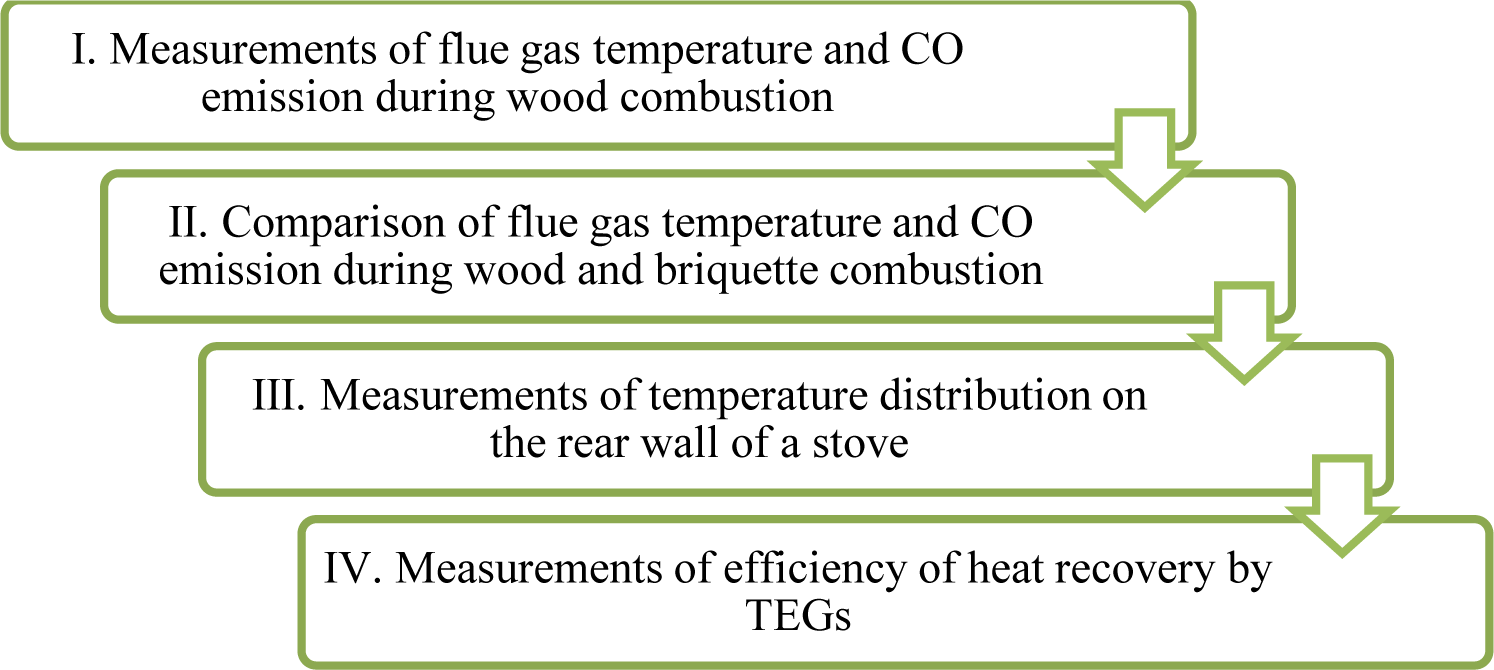
In Scenario I (basic scenario), the process of combustion of subsequent inputs of spruce logs (further in the text as ‘wood’) was presented. The process was divided into three main phases: ignition phase (when 2.5 kg of the wood was combusted to preheat the combustion chamber), combustion phase (when two inputs of 2.5 kg and two inputs of 3.0 kg were combusted), and afterburning phase (the final phase of the combustion process). This scenario corresponds to the typical way of using the tested stove (except for the lack of air throttle opening control, which was fully opened during the entire combustion process). In Scenario II, wood and beech-birch briquet (further in the text as ‘briquet’) were combusted during the following iterations of combustion experiments. The fuel load was 2.5 – 3.0 kg in the case of wood combustion and 4.5 kg in the case of briquette combustion (the highest mass of briquette resulted from its higher bulk density). The following parameters were measured: flue gas temperature and emissions of CO, CO2, O2, NOx and SO2. Moreover, the PM emission was also gravimetrically measured according to Szramowiat-Sala et al., 2019 [32] and the samples of PM were analytically treated to determine the content of benzo-a-pirene (BaP) according to Szramowiat-Sala et al., 2023 [3]. The results of Scenario I and Scenario II allowed to assess the durability of the combustion process and select the best scenario for the most effective heat recovery by a TEG mounted in further analysis. Scenario III was conducted to investigate the thermographic performance of a manually operated stove, which was integrated with thermoelectric generators, according to Figure 2. In Scenario IV, the efficiency of power generation by TEGs was finally evaluated. During the experiments, the following operation parameters were measured: flue gas temperature in the furnace (tfg1), flue gas temperature at the outlet of the stove (tfg2), rear wall temperature (text), ambient air temperature (tair), the cooling water temperature at the inlet and outlet of the water-cooled TEG (tw1, tw2), the cooling water flow (mw) as well as current (ITEG), voltage (VTEG) and power (PTEG) generated in TEG1 and TEG2. Moreover, temperature distribution on the rear wall was measured using an infrared camera (IR). All data (except PM and thermography analysis) was processed via a control and measurement system with the WAGO PFC200 PLC controller (PLC). The regulation of the air flowing to the furnace was possible using a throttle with a special servomechanism. The stove operation was controlled using a dedicated algorithm implemented in the CoDeSys software. The location of the measurement points is shown in Figure 4.
The location of the measurement points
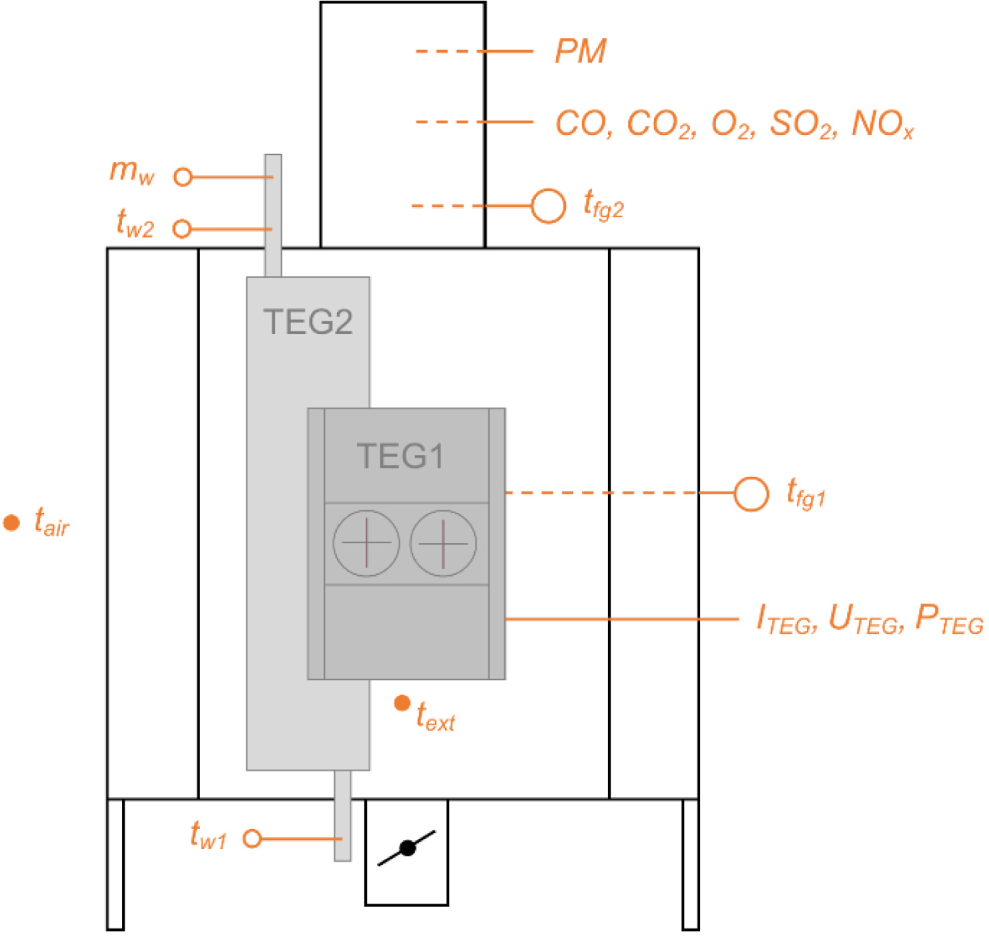
To replicate real-life conditions of wood-fired stoves, both the stove and the combustion process, including ignition, loading, etc., were manually controlled and operated. This hands-on approach aimed to simulate practical scenarios accurately and ensure the reliability and relevance of the experimental results.
This section presents the most important findings resulting from the conducted works. As was presented in Figure 3, it includes the following parts: (i) measurements of flue gas temperature and CO emission during wood combustion, (ii) comparison of flue gas temperature and CO emission during wood and briquette combustion, (iii) measurements of temperature distribution on the rear wall of a stove, and (iv) measurements of efficiency of heat recovery by thermoelectric generators.
The basic operating scenario corresponds to the typical use of the tested stove. As can be observed in Figure 5, during the combustion phase, the maximum flue gas temperature (tfg2) reached the level of 283.5 °C (when 2.5 kg of wood were combusted) and 317.5 °C (when 3.0 kg of wood were combusted). On the other hand, significant fluctuations in flue gas temperature were observed. The lower flue gas temperature in time, when the subsequent fuel inputs were added, dropped to approximately 100 °C. Consequently, the average value of flue gas temperature during the combustion phase varied from 191.3 °C (when fuel inputs 1 and 2 were realized) to 202.2 °C (when fuel inputs 3 and 4 were realized). Considering the observed variations in flue gas temperature, it may be concluded that the combustion process can be repeatable when the same operating conditions are provided (including fuel inputs and a level of air throttle opening).
Variations in flue gas temperature during the combustion process – scenario I
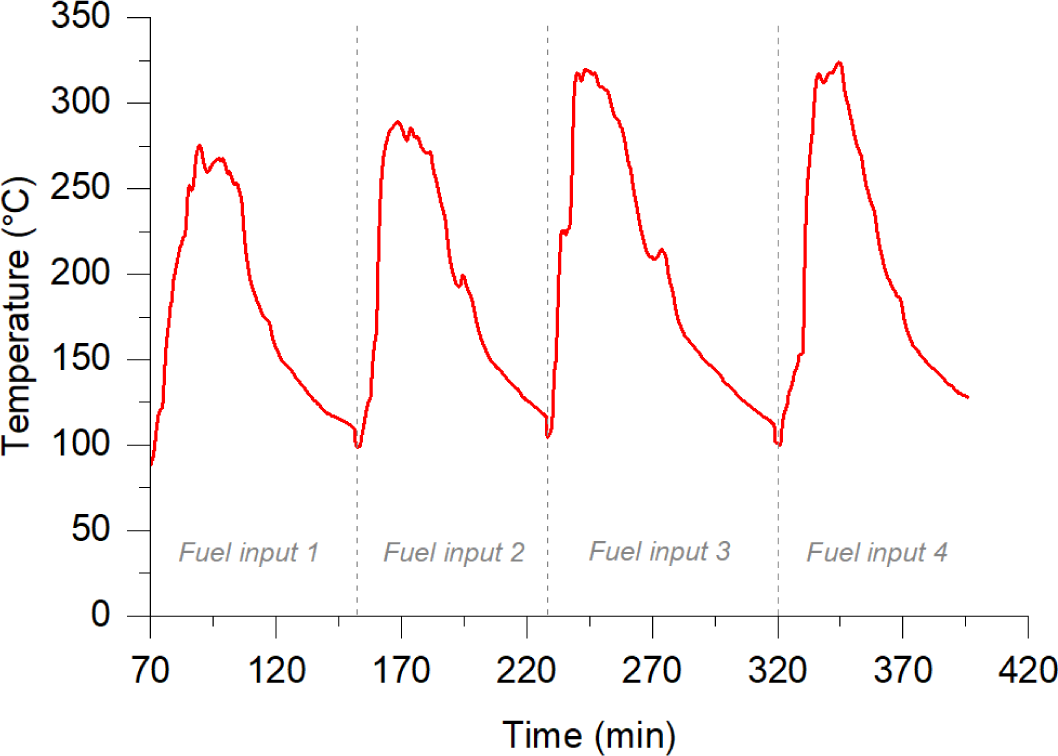
Apart from flue gas temperature variations, carbon monoxide emissions were observed during the analyzed combustion process. As shown in Figure 6, CO emissions varied from 350 mg/m3 (in time, when the highest temperature of flue gas occurred) to 8200 mg/m3 (in time, when the subsequent fuel inputs were realized). However, the average value of CO emission during the combustion phase in the analyzed combustion process was 2888 mg/m3. This value is significantly higher than the maximum value given by Ecodesign, but it is connected, e.g., with the combustion parameters (including using a fully opened air throttle, while in typical conditions, it is controlled using the temperature of the flue gas).
Variations in carbon monoxide emission during the combustion process – scenario I
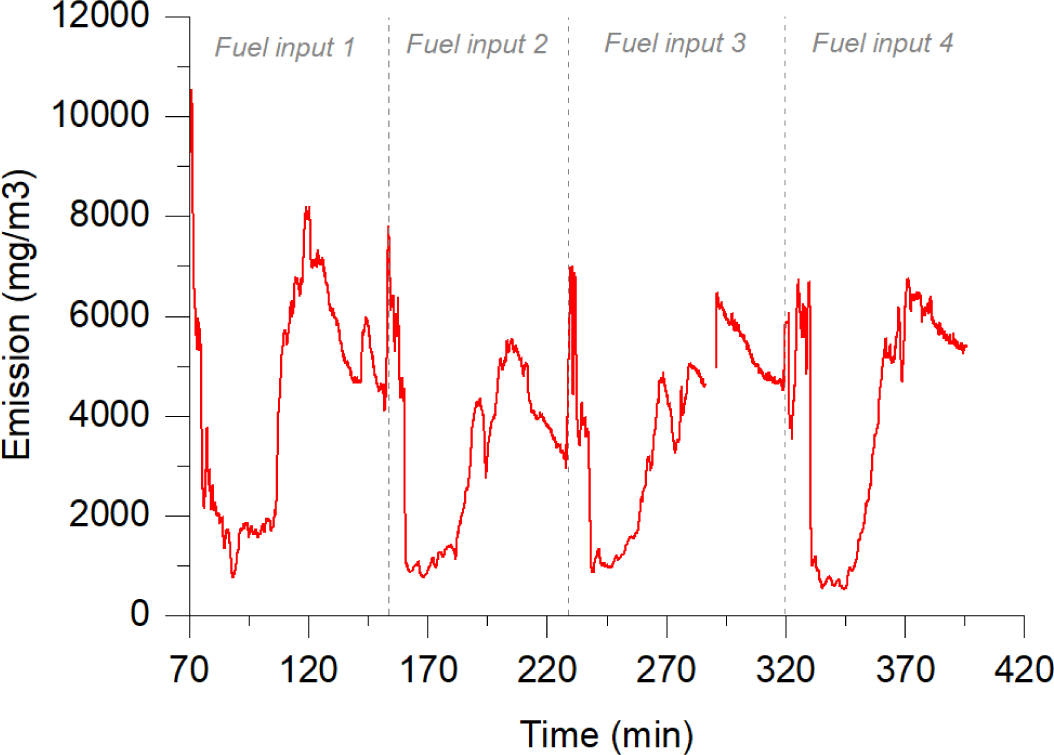
Figure 7 illustrates the temperature variations of the flue gas during the combustion of 2.5 kg of wood and 4.5 kg of briquet. Consequently, the results show that the highest flue gas temperature was achieved when burning 4.5 kg of briquet, registering a 9.7% increase compared to the maximum temperature during wood combustion (for the stove without an accumulation layer). However, with the implementation of an accumulation layer, a substantial difference in flue gas temperatures was observed. Compared to wood combustion, the maximum temperature during briquet combustion was higher by approximately 43.5%. This indicates that the accumulation layer significantly impacted combustion, producing higher flue gas temperatures during briquet burning.
Variations in the flue gas temperature tfg2
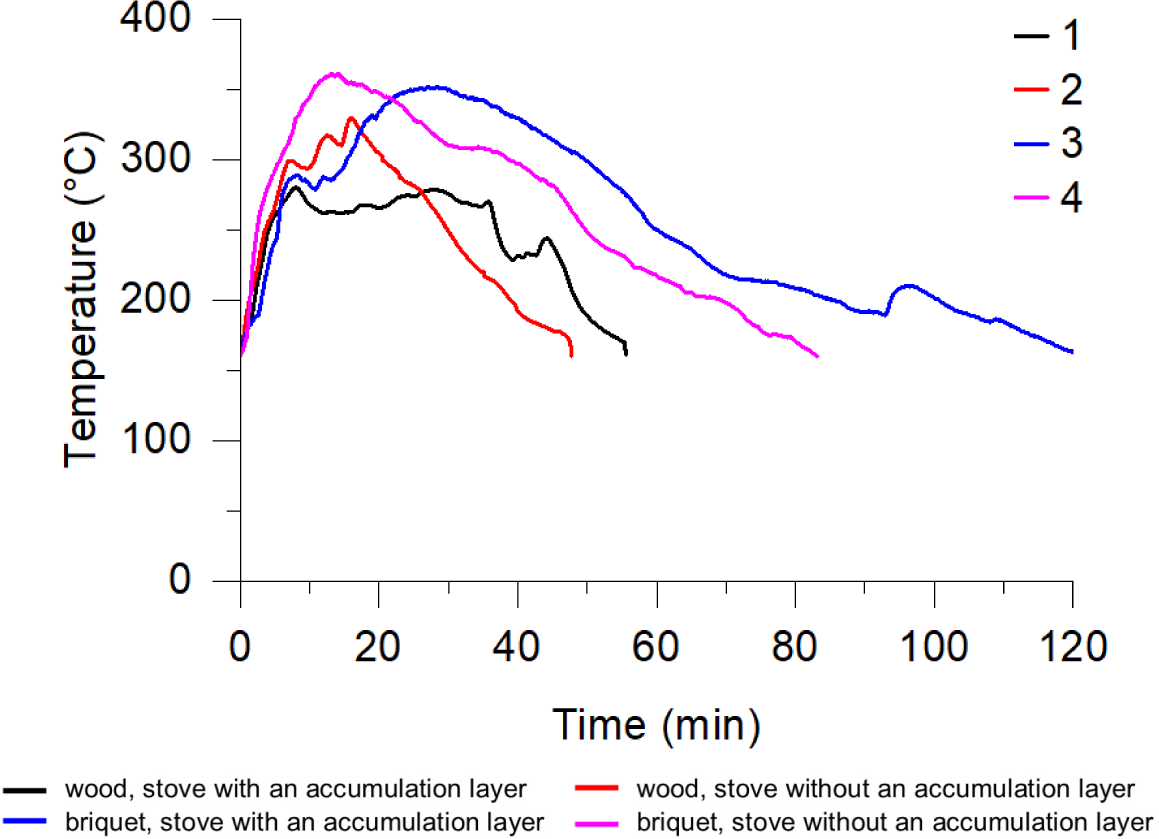
Moreover, the combustion process duration varied significantly depending on the specific combustion conditions. For instance, the wood combustion in the stove without an accumulation layer lasted approximately 38 minutes. Conversely, when the briquet was burned in the stove equipped with an accumulation layer, the combustion process extended to approximately 122 minutes. These variations highlight the influence of both the fuel type and the presence of an accumulation layer on the duration of the combustion process.
Overall, these findings emphasize the importance of considering fuel type and the utilization of accumulation layers when assessing temperature variations and combustion process durations in wood-fired stoves. Such information is crucial for further analysis of the implementation of the TEG concept.
Furthermore, assessing and optimizing emission performance is crucial to minimizing environmental impact, adhering to emission standards, and enhancing air quality. Figure 8 illustrates the variations in CO emissions during the combustion of 5 kg of wood and briquet. The CO emission significantly fluctuated throughout the combustion processes.
Variations in carbon monoxide emission
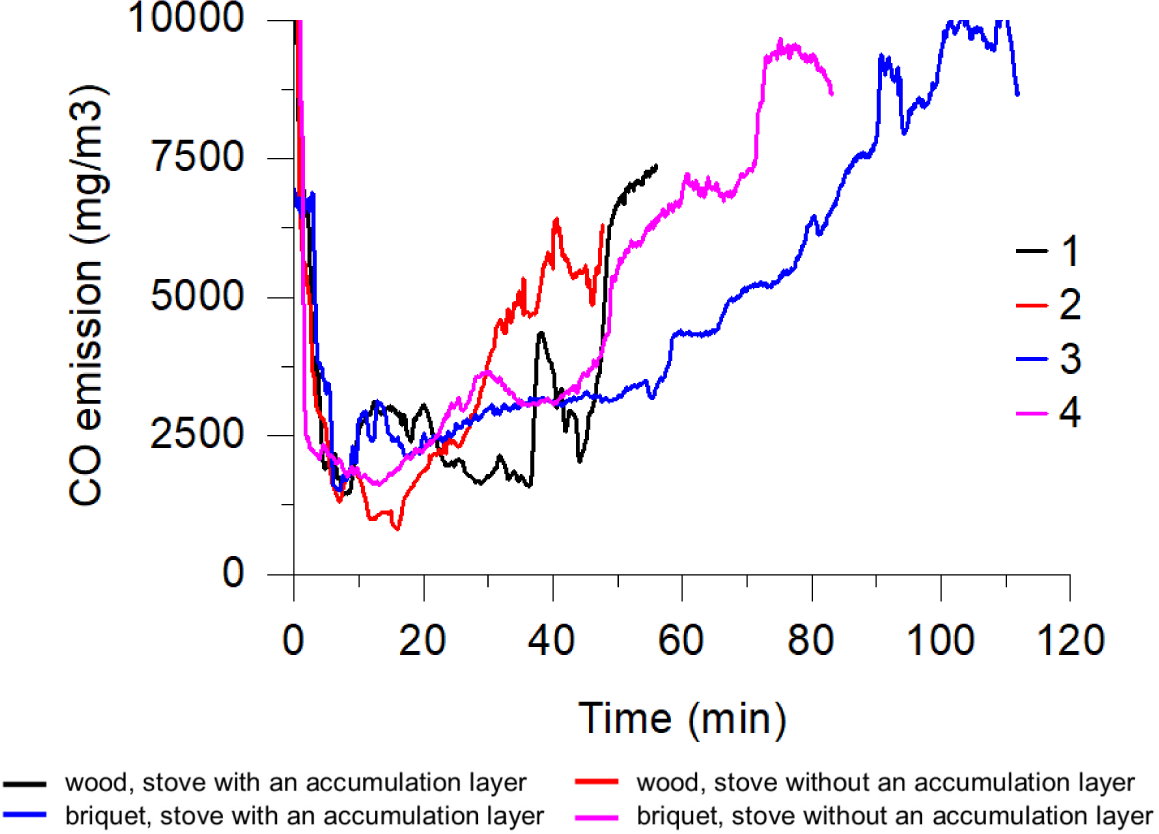
Upon analyzing the collected data, it was observed that the lowest CO emission occurred during wood combustion in the stove without an accumulation layer. The average CO emission value during this phase (determined when the flue gas temperature exceeded 250 °C and adjusted to 13% O2) was 1,916 mg/m3. However, it is essential to note that this value surpasses the Eco-Design requirements, which limit CO emission for room heaters to 1,500 mg/m3.
Contrastingly, the average CO emission during other combustion series was even higher, reaching a maximum level of 2,904 mg/m3 when the briquet was burned in the stove equipped with an accumulation layer. Although the average concentration of carbon monoxide (CO) was highest when burning briquet in a stove with an accumulation layer, the rate of CO release during the combustion process was slower than other tests. For around 75% of the combustion duration, the amount of CO released was the lowest among all scenarios tested. These findings highlight the impact of different combustion conditions, fuel types, and the presence of accumulation layers on CO emissions.
The CO emission levels observed in these wood-fired stoves raise concerns about compliance with environmental regulations and the potential health implications of higher pollutant concentrations. It emphasizes the need for cleaner and more efficient wood combustion technologies and the importance of adhering to emission standards for promoting healthier indoor and outdoor air quality.
Table 2 shows the averaged values of gaseous components in the flue gas, along with particulate matter (PM) and PM-bound BaP. We discovered that the concentration of almost every parameter measured increased when the accumulation layer was applied, except for BaP and CO2. The increased concentrations of gaseous components in the flue gas (excluding BaP and CO2) after implementing the accumulation layer in wood-fired stoves without automatic operation suggest that adding the layer might have influenced the combustion process, leading to higher emissions of certain gases. The reduction in CO2 levels can be explained by the equivalence of the conversion of CO to CO2 during combustion [33]. When the CO2 reduction was observed, the increase in CO concentration was recorded as well. The decrease in BaP concentration indicates that the accumulation layer's intended effect of raising the combustion chamber's temperature was successful either in reducing the emissions of this component of particulate matter or in converting BaP bound with PM into gaseous form [34]. Overall, these findings highlight the complexity of the combustion process and the potential impact of implementing accumulation layers on emission profiles in wood-fired stoves. Additionally, it is essential to synchronize efforts in improving stove performance by focusing on enhanced device types, optimized operation, automation, and utilizing high-quality fuels [35].
Average emission of flue gas gaseous and solid components during wood and briquet combustion
Emission parameter measured |
A stove WITH accumulation layer |
A stove WITHOUT accumulation layer |
---|---|---|
WOOD |
||
CO [mg/m3, 13% O2] |
2,227 |
1,916 |
CO2 [%] |
3.2 |
3.8 |
O2 [%] |
17.9 |
17.3 |
NOx [ppm] |
53.0 |
62.3 |
SO2 [ppm] |
26.3 |
15.4 |
PM [mg/m3] |
17.9 |
16.71 |
BaP [µg/kg] |
1.33 |
2.25 |
BRIQUET |
||
CO [mg/m3, 13% O2] |
2,904 |
2,812 |
CO2 [%] |
3.8 |
4.0 |
O2 [%] |
17.3 |
17.0 |
NOx [ppm] |
98.5 |
53.7 |
SO2 [ppm] |
43.9 |
23.9 |
PM [mg/m3] |
3162.5 |
2644.6 |
BaP [µg/kg] |
- |
- |
To assess the influence of an accumulation layer on the emission performance of a wood-fired stove, the changes in particulate matter (PM) released during fuel-burning phases, including ignition and combustion, were investigated. Figure 9 compares the variations of PM emissions during these burning phases between a stove with and without the accumulation layer. Gravimetric measurements of PM were taken after loading 2.5 kg and 3.0 kg of wood during the ignition and combustion phases, respectively. The results showed that when an accumulation layer was applied, a decreasing trend in PM concentration was observed throughout the phases of wood burning, suggesting a potential positive impact on emission reduction. In contrast, when the stove operated without an accumulation layer, a notable sudden increase in PM concentration was observed towards the end of the combustion phase.
Emission of particulate matter during wood combustion in a stove with and without accumulation layer
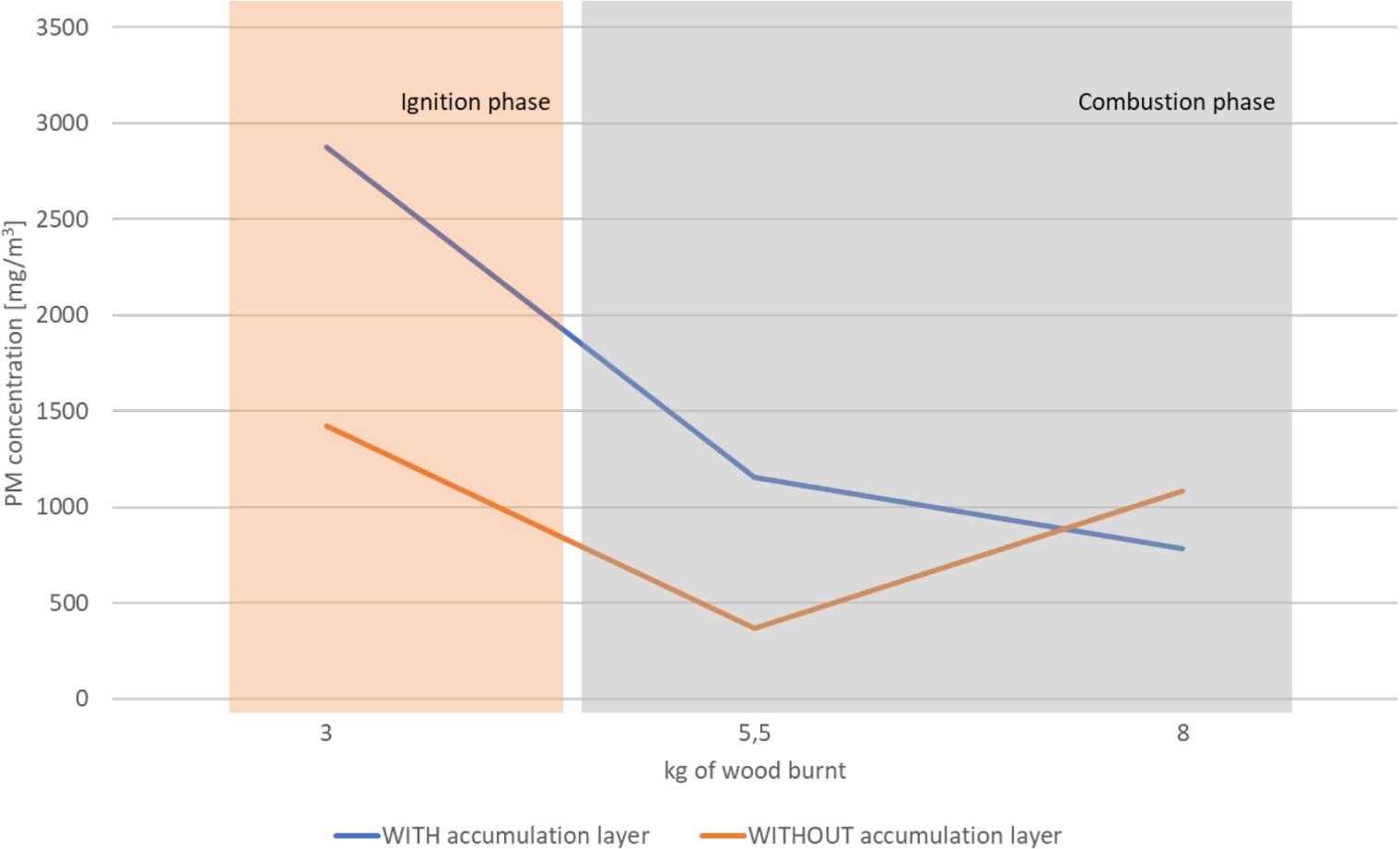
Based on the superior temperature performance observed during briquet burning in a stove with an accumulation layer, we further investigated the correlation between briquet loads during specific combustion phases (combustion and afterburning) and particulate matter (PM) emissions to analyze the impact of manual operation (see Figure 10).
Variation of particulate matter emission depending on the load of briquet burnt in a stove with an accumulation layer
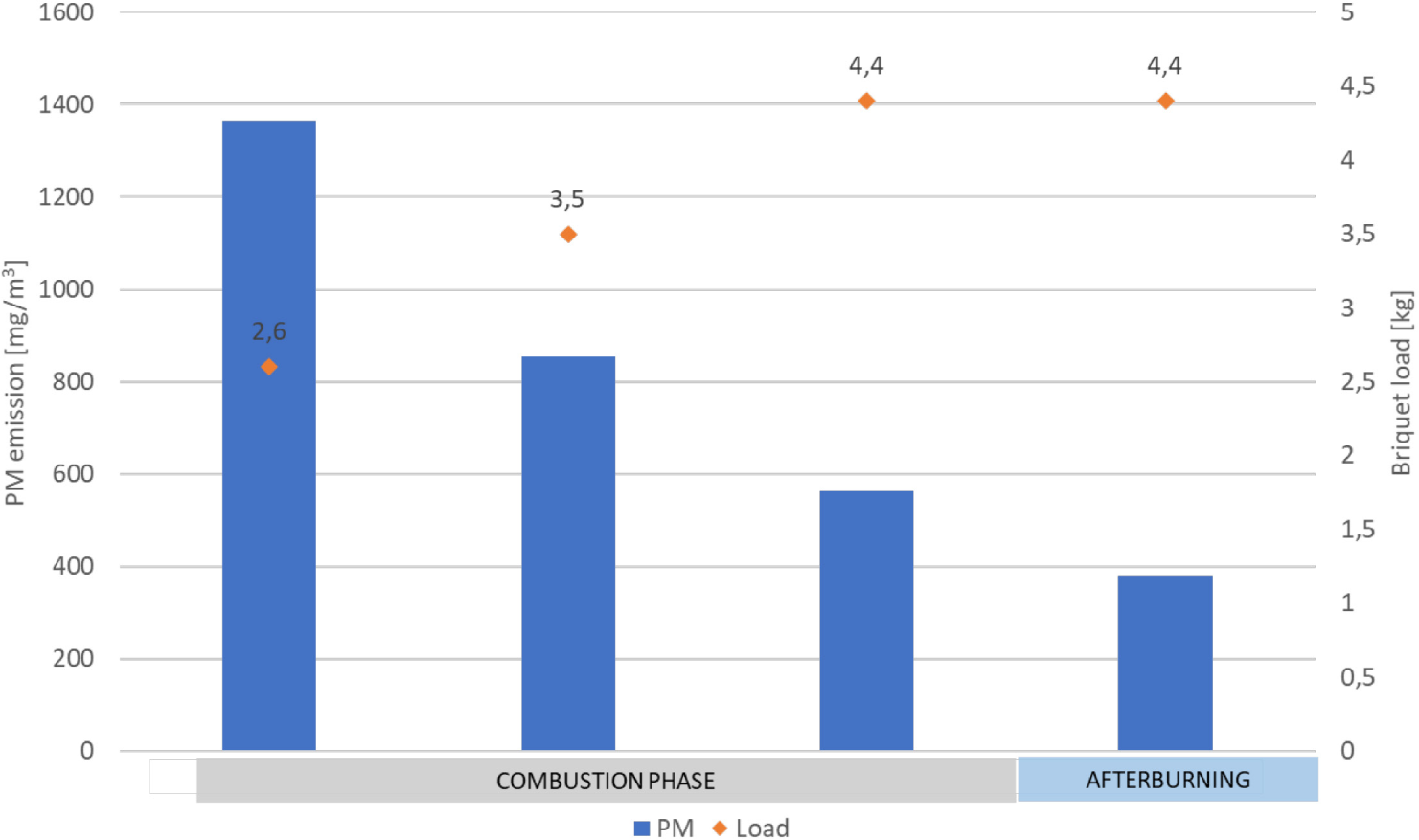
The results revealed that as the briquet load increased, PM emissions decreased, and the afterburning phase exhibited the lowest PM concentration. Briquets, being an eco-friendly fuel specifically designed for environmental sustainability, play a crucial role in reducing greenhouse gas and pollutant emissions from domestic combustion. Therefore, to achieve optimal temperature conditions for mounting thermoelectric generators while ensuring ecological parameters such as optimal CO performance and reduced PM throughout the combustion phases, briquet loads of 4.5 kg were selected for subsequent scenarios (Scenario III and Scenario IV) of the experiments. This strategic decision aims to strike a harmonious balance between energy efficiency and ecological considerations in the operation of wood-fired stoves.
Based on the conclusions from Scenario I and II in the following iterations of experiments, briquet (4.5 kg load) was combusted in a stove with and without an accumulation layer.
From the perspective of using a thermoelectric generator, the temperature at the rear wall plays a crucial role. This data is essential for understanding the temperature distribution and optimizing the thermoelectric generator's performance during the combustion process. As depicted in Figure 11, the presence of the accumulation layer limited the temperature in the corresponding area to a maximum of 120 °C. In contrast, the deflector area experienced much higher temperatures, reaching up to 230 °C. A thermal image was taken to capture the moment when the temperature at the rear wall (measured at point tout) reached its peak value.
Temperature distribution on the rear wall of the tested stove WITH accumulation layer
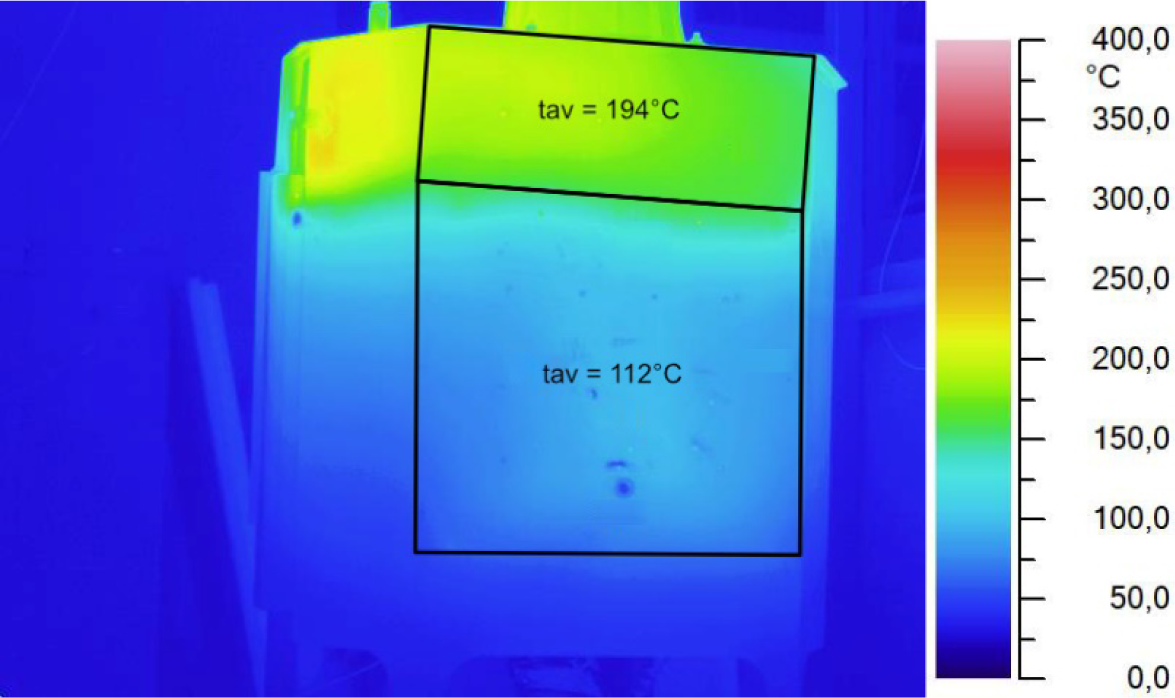
Conversely, upon removing the accumulation layer, the temperature of the rear wall significantly increased, reaching levels approximately 3-4 times higher than the previous observation. The highest temperature recorded exceeded 500 °C, but this extreme temperature was limited to a small region in the central lower part of the stove's rear wall.
The cooling system of the TEGs contributed to heat dissipation, resulting in a lower temperature near the TEGs compared to the surrounding area, regardless of whether the accumulation layer was present or not. Additionally, the installation of TEG2 led to approximately 30% of its hot surface being exposed to temperatures below 220 °C, as depicted in Figure 12. This specific positioning of TEG2 in the lower temperature area highlights the variability in heat distribution and the need to consider TEG placement for optimal performance.
Temperature distribution on the rear wall of the tested stove WITHOUT accumulation layer and without thermoelectric generator installed (a), as well as with a thermoelectric generator 1 (b) and a thermoelectric generator 2 (c) installed, respectively
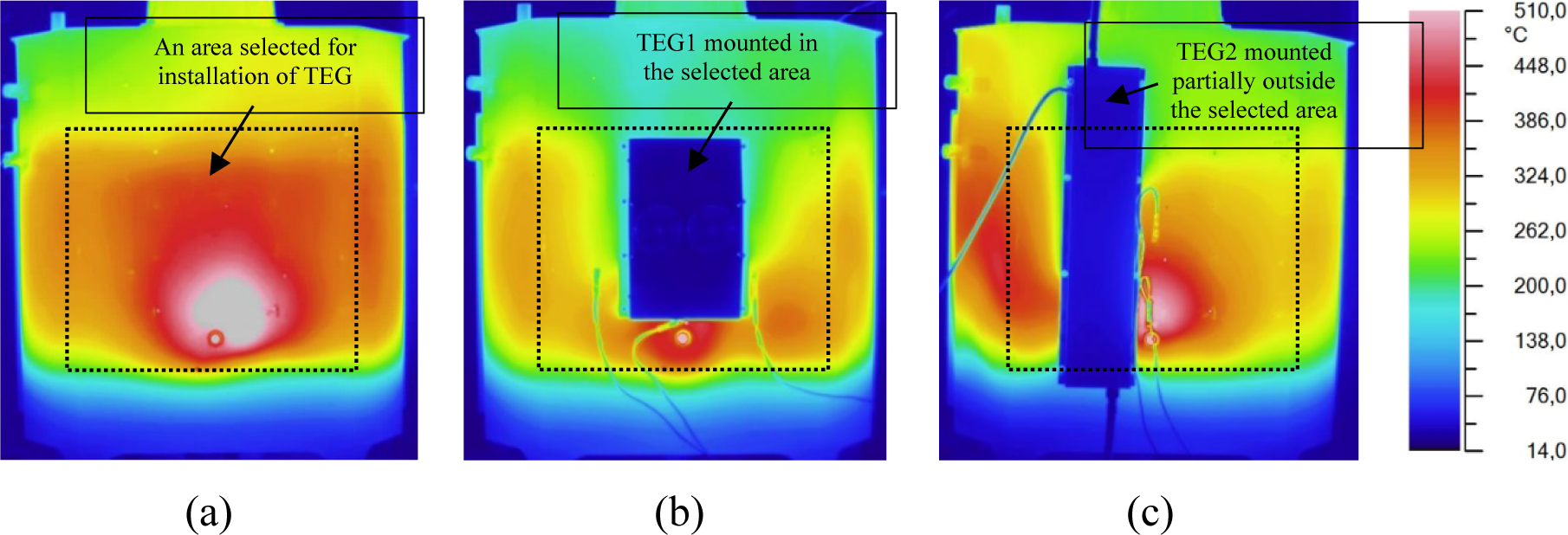
Understanding these temperature fluctuations and heat distribution patterns is crucial for effectively utilizing thermoelectric generators and enhancing their efficiency during the combustion process. Proper consideration of TEG positioning and monitoring temperature changes will aid in maximizing the energy conversion capabilities of the system and ensuring its overall effectiveness.
The achieved temperature level and uniformity on the stove's rear wall significantly impacted the tested thermoelectric generators power generation. In Figure 13 and Figure 14, the current-voltage (I-V) and power-voltage (P-V) characteristics of TEG1 and TEG2 are presented, respectively. These characteristics were obtained at four different temperature values, measured in the central part of the stove's rear wall (tout).
The variations in voltage and current generated by TEG1, as depicted in Figure 13a, demonstrated an increase in open-circuit voltage, short-circuit current, and voltage and current at specific measurement points with a rise in the stove's rear wall temperature. These variations were influenced by factors such as the combustion phase, fuel input, and the continuity of the combustion process. The highest short-circuit current measured was 4.4 A, and the open-circuit voltage reached 16.5 V when the external wall temperature was at 380 °C.
Temperature variations also played a significant role in the power curves, as shown in Figure 13b. The graph illustrates the fluctuation in the power collected from the thermoelectric generator concerning voltage. The maximum power achieved was 18.8 W at a current of 2.3 A and a voltage of 8.2 V. However, this value represented only 41.7% of the nominal power of the tested TEG1. The reduced power output was influenced by lower than required hot side temperature and its nonuniform distribution on the rear wall, as observed in Figure 12a. Additionally, heat dissipation by the TEG cooling system may have contributed to this situation.
Current-voltage (a) and power-voltage (b) characteristics of the thermoelectric generator 1 for the various temperatures of the rear wall
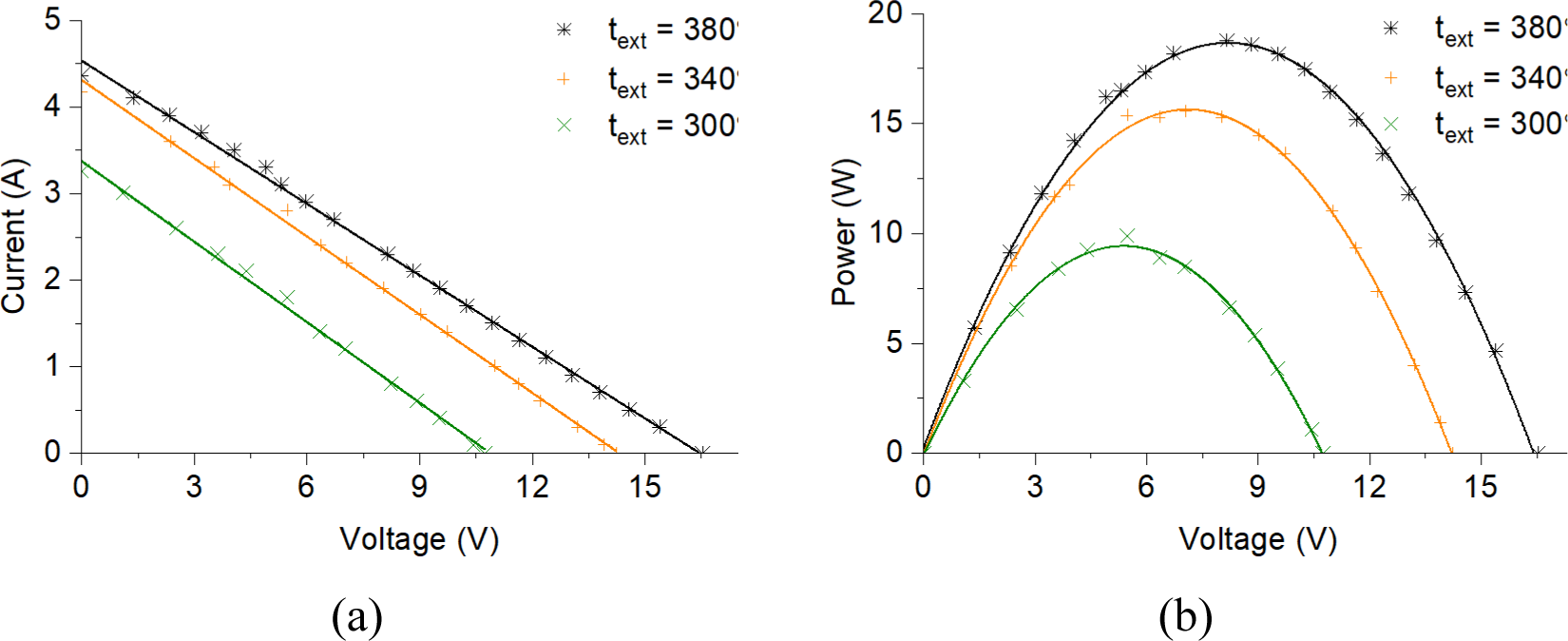
Mounting TEG2 presented more challenges due to the limited available area on the stove's rear wall. Only around 70% of the TEG2's hot surface was positioned in an area with sufficient temperature to power it. Consequently, the short-circuit current did not exceed 5.25 A, and the open-circuit voltage did not surpass 24.1 V (see Figure 14). Although TEG2 generated higher power than TEG1, the value of 31.2 W represented only 31.2% of the nominal power. This performance difference may be attributed to the limitations in positioning TEG2, which affected its overall power generation capacity.
Current-voltage (a) and power-voltage (b) characteristics of the thermoelectric generator 2 for the various temperatures of the rear wall
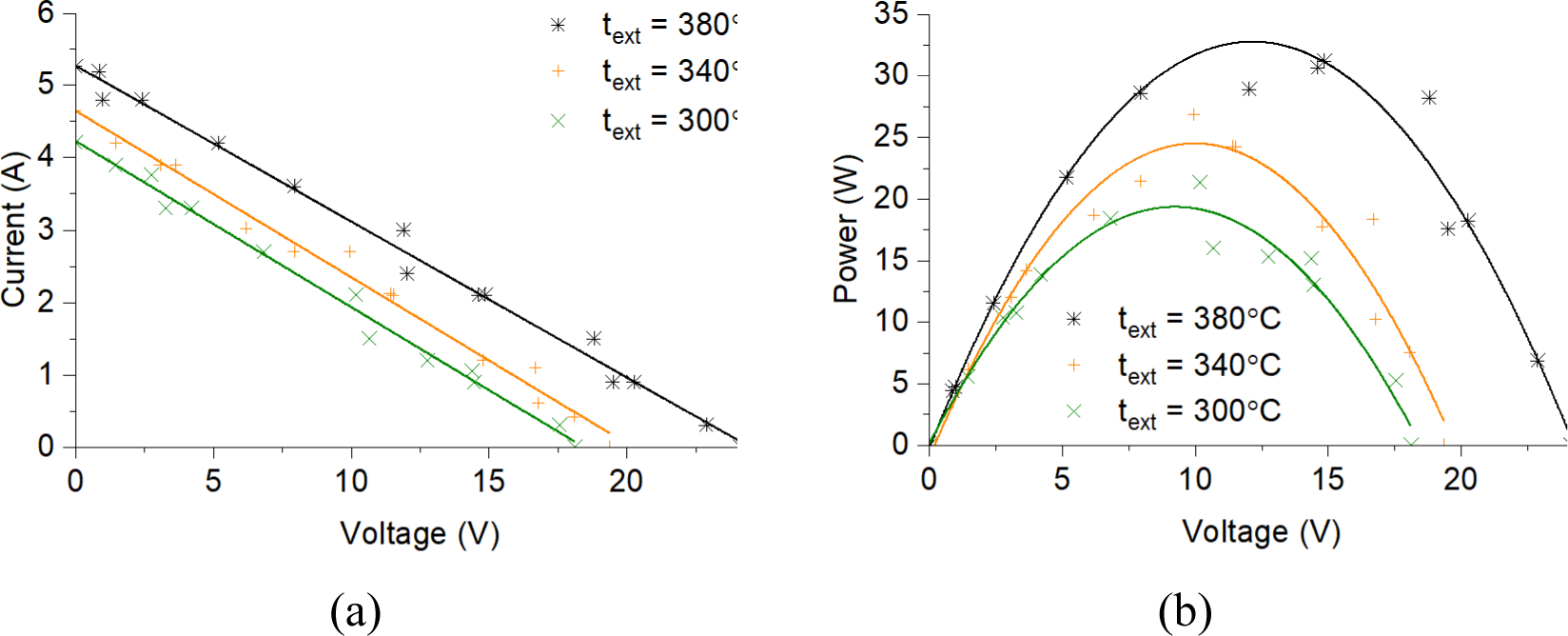
In conclusion, the temperature variations and distribution on the rear wall of the stove significantly influenced the power generation capabilities of the tested thermoelectric generators. Proper placement and uniform temperature distribution are crucial factors to consider for maximizing the performance of TEGs and harnessing their full potential in wood-fired stove applications. Despite the fact that tested thermoelectric generators produced reduced power in comparison to their nominal capacity, they are competitive with other examples presented in the literature (see Table 3).
The comparison of obtained results with results of selected previous research on the thermoelectric generators integrated with stoves
Heat Source |
Power, We |
Application |
Development Status |
Source |
---|---|---|---|---|
Heating stove |
18.8 |
|
Laboratory tests |
this paper |
Cooking stove |
2.4 |
Battery charging |
Laboratory tests |
[36] |
Cooking stove |
5.0 |
Battery charging and lighting |
Laboratory tests |
[37] |
Multifunction stove |
9.5 |
Battery charging |
Laboratory tests |
[38] |
Portable gas stove |
12.9 |
External load |
Laboratory tests |
[39] |
Solid-fuel stove |
27.0 |
Battery charging and power other equipment |
Laboratory tests |
[40] |
Cooking stove |
5.9 |
Battery charging and power other equipment |
Field tests |
[41] |
Multifunction stove |
28.0 |
Power stove’s fan |
Laboratory tests |
[42] |
Thermoelectric generators show promising potential as energy sources alongside home heating devices. However, their proper selection and complex usage pose challenges. Factors like operating and maximum hot side temperatures and required cold side temperature greatly influence TEG performance.
The research was conducted in four stages allowed us to identify the most favorable conditions for integrating TEGs with manually operated wood-fired stoves. In Scenario I (basic scenario), the process of combustion of subsequent inputs of spruce logs was presented. The process was divided into three main phases: ignition phase (when 2.5 kg of the wood was combusted to preheat the combustion chamber), combustion phase (when two inputs of 2.5 kg and two inputs of 3.0 kg were combusted), and afterburning phase (the final phase of the combustion process). In Scenario II, wood and beech-birch briquet were combusted during the following iterations of combustion experiments. The fuel load was 2.5 – 3.0 kg in the case of wood combustion and 4.5 kg in the case of briquette combustion (the highest mass of briquette resulted from its higher bulk density). The results of Scenario I and Scenario II allowed to assess the durability of the combustion process and identify the most optimal conditions for the most effective heat recovery by a TEG mounted in further analysis, specifically, briquet combustion in a stove without an accumulation layer. While the accumulation layer is intended to enhance the combustion process by storing and gradually releasing heat to the indoor environment, it inadvertently hinders waste heat input to the TEGs, resulting in poorer energy performance than stoves without accumulation layers. Scenario III was conducted to investigate the thermographic performance of a manually operated stove, which was integrated with thermoelectric generators. In Scenario IV, the efficiency of power generation by TEGs was finally evaluated. During our tests, TEG1 generated only 18.8 W (compared to its 45 W nominal power), and TEG2 produced 31.2 W (compared to its 100 W nominal power). The low output can be attributed to suboptimal stove and TEGs integration.
These findings underscore the significance of considering thermal and ecological efficiency when designing and using wood-fired stoves. Adding an accumulation layer can aid in achieving optimal combustion conditions, ultimately leading to improved energy efficiency and decreased emissions of harmful substances into the atmosphere. By addressing integration challenges and optimizing stove-TEG connections, we can unlock the full potential of thermoelectric generators in enhancing the performance of wood-fired stoves and promoting sustainable heating solutions.
This work was carried out under Subsidy from the Faculty of Energy and Fuels, AGH University of Science and Technology in Krakow (no. 16.16.210.476). The Center of Energy, AGH UST infrastructure in Krakow was used. The research project was partly supported by the “Excellence Initiative – Research University” program for the AGH University of Science and Technology.
ITEG |
current |
[A] |
mw |
the cooling water flow |
[kg/s] |
PTEG |
power |
[W] |
tfg1 |
flue gas temperature in the furnace |
[°C] |
tfg2 |
flue gas temperature at the outlet of the stove |
[°C] |
text |
rear wall temperature |
[°C] |
tair |
ambient air temperature |
[°C] |
tw1 |
the cooling water temperature at the inlet to the water-cooled TEG |
[°C] |
tw2 |
the cooling water temperature at the outlet of the water-cooled TEG |
[°C] |
VTEG |
voltage |
[V] |
Abbreviations |
||
AC |
Alternating Current |
|
BaP |
Benzoapyrene |
|
DC |
Direct Current |
|
HC |
Hydrocarbons |
|
IR |
Infrared camera |
|
OGC |
Organic Gas Compounds |
|
PAHs |
Polycyclic Aromatic Hydrocarbons |
|
PLC |
Programmable Logic Controller |
|
PM |
Particulate Matter |
|
TEGs |
Thermoelectric Generators |
|
μCHP |
Micro-Cogeneration Systems |
|
VOCs |
Volatile Organic Compounds |
Techno-economic, Social and Environmental Assessment of Biomass Based District Heating in a Bioenergy Village ,Journal of Sustainable Development of Energy, Water and Environment Systems , Vol. 7 (4),pp 601-614 , 2019, https://doi.org/https://doi.org/10.13044/J.SDEWES.D7.0257
, Thermogravimetric and Kinetic Analysis of Waste Biomass and Plastic Mixtures ,Journal of Sustainable Development of Energy, Water and Environment Systems , Vol. 11 (4),pp 1110469 , 2023, https://doi.org/https://doi.org/10.13044/j.sdewes.d11.0469
, The properties of particulate matter generated during wood combustion in in-use stoves ,Fuel , Vol. 253 ,pp 792–801 , 2019, https://doi.org/https://doi.org/10.1016/j.fuel.2019.05.026
, Heavy Metals in Air Nanoparticles in Affected Industry Area ,Journal of Sustainable Development of Energy, Water and Environment Systems , Vol. 5 (1),pp 58–68 , 2017, https://doi.org/https://doi.org/10.13044/j.sdewes.d5.0133
, A review of the physicochemical characteristics of ultrafine particle emissions from domestic solid fuel combustion during cooking and heating ,Science of The Total Environment , Vol. 886 ,pp 163747 , 2023, https://doi.org/https://doi.org/10.1016/J.SCITOTENV.2023.163747
, Emission characteristics of modern and old-type residential boilers fired with wood logs and wood pellets ,Atmos Environ , Vol. 38 (25),pp 4183–4195 , 2004, https://doi.org/https://doi.org/10.1016/J.ATMOSENV.2004.04.020
, Fine particulate matter and gas emissions at different burn phases from household coal-fired heating stoves ,Atmos Environ , Vol. 305 ,pp 119803 , 2023, https://doi.org/https://doi.org/10.1016/J.ATMOSENV.2023.119803
, Chemical composition and speciation of particulate organic matter from modern residential small-scale wood combustion appliances ,Science of the Total Environment , Vol. 612 ,pp 636–648 , 2018, https://doi.org/https://doi.org/10.1016/j.scitotenv.2017.08.263
, Light my fire but don’t choke on the smoke: Wellbeing and pollution from fireplace use in Sweden ,Energy Res Soc Sci , Vol. 69 ,pp 101696 , 2020, https://doi.org/https://doi.org/10.1016/J.ERSS.2020.101696
, - , Implementing Directive 2009/125/EC of the European Parliament and of the Council of 21 October 2009 with regard to ecodesign requirements for solid fuel local space heaters., 2015
Multi-Objective Target-Oriented Robust Optimization of Biomass Co-Firing Networks Under Quality Uncertainty ,Journal of Sustainable Development of Energy, Water and Environment Systems , Vol. 9 (2),pp 1080364 , 2021, https://doi.org/https://doi.org/10.13044/j.sdewes.d8.0364
, Conversion of lignocellulose biomass to bioenergy through nanobiotechnology ,Journal of Sustainable Development of Energy, Water and Environment Systems , Vol. 11 (2),pp 1110442 , 2023, https://doi.org/https://doi.org/10.13044/j.sdewes.d11.0442
, Feasibility study for water-electricity cogeneration using integrated system of concentrated solar power and biofuel as renewable energy sources ,Journal of Sustainable Development of Energy, Water and Environment Systems , Vol. 9 (3), 2021, https://doi.org/https://doi.org/10.13044/J.SDEWES.D8.0372
, Modelling and Control of Collecting Solar Energy for Heating Houses in Norway ,Journal of Sustainable Development of Energy, Water and Environment Systems , Vol. 5 (3),pp 359–376 , 2017, https://doi.org/https://doi.org/10.13044/j.sdewes.d5.0147
, Artificial Intelligence in Environmental Monitoring: Application of Artificial Neural Networks and Machine Learning for Pollution Prevention and Toxicity Measurements , 2023, https://doi.org/https://doi.org/10.20944/PREPRINTS202307.1298.V1
, Integration of Water and Energy Planning to Promote Sustainability ,Journal of Sustainable Development of Energy, Water and Environment Systems , Vol. 7 (2),pp 229–252 , 2019, https://doi.org/https://doi.org/10.13044/j.sdewes.d6.0246
, Methods for Assessing Energy Efficiency of Buildings ,Journal of Sustainable Development of Energy, Water and Environment Systems , Vol. 7 (3),pp 432–443 , 2019, https://doi.org/https://doi.org/10.13044/j.sdewes.d6.0243
, Optimization Methods for Energy Consumption Estimation in Wireless Sensor Networks ,Journal of Sustainable Development of Energy, Water and Environment Systems , Vol. 7 (2),pp 261-274 , 2019, https://doi.org/https://doi.org/10.13044/j.sdewes.d6.0244
, Comparison of Data-Driven Thermal Building Models for Model Predictive Control ,Journal of Sustainable Development of Energy, Water and Environment Systems , Vol. 7 (4),pp 730-742 , 2019, https://doi.org/https://doi.org/10.13044/j.sdewes.d7.028
, Intelligent Algorithm for Efficient Use of Energy Using Tackling the Load Uncertainty Method in Smart Grid ,Journal of Sustainable Development of Energy, Water and Environment Systems , Vol. 8 (3),pp 547-560 , 2020, https://doi.org/https://doi.org/10.13044/j.sdewes.d7.0308
, Application of Statistical and Artificial Intelligence Techniques for Medium-Term Electrical Energy Forecasting: A Case Study for a Regional Hospital ,Journal of Sustainable Development of Energy, Water and Environment Systems , Vol. 8 (3),pp 520-536 , 2020, https://doi.org/https://doi.org/10.13044/j.sdewes.d7.0306
, Optimal Capacities of Distributed Renewable Heat Supply in a Residential Area Connected to District Heating ,Journal of Sustainable Development of Energy, Water and Environment Systems , Vol. 9 (1),pp 1080328 , 2021, https://doi.org/https://doi.org/10.13044/j.sdewes.d8.0328
, Method to Model the Hourly Variability of Renewable Energy Sources in Integrated Assessment Models ,Journal of Sustainable Development of Energy, Water and Environment Systems , Vol. 12 (1),pp 1110481 , 2024
, Analysis of the use of different standards for estimation of energy efficiency measures in the building sector ,Journal of Sustainable Development of Energy, Water and Environment Systems , Vol. 10 (1),pp 1080375 , 2022, https://doi.org/https://doi.org/10.13044/j.sdewes.d8.037
, Assessment of Waste Heat Recovery in the Steel Industry ,Journal of Sustainable Development of Energy, Water and Environment Systems , Vol. 11 (2),pp 1100440 , 2023, https://doi.org/https://doi.org/10.13044/j.sdewes.d10.0440
, Effect of Process Parameters on Bioelectricity Production, Energy and Environmental Performance ,Journal of Sustainable Development of Energy, Water and Environment Systems , Vol. 7 (4),pp 567-583 , 2019, https://doi.org/https://doi.org/10.13044/j.sdewes.d6.0237
, Thermoelectricity - A Promising Complementarity with Efficient Stoves in Off-grid-areas ,Journal of Sustainable Development of Energy, Water and Environment Systems , Vol. 3 (3),pp 256-268 , 2015, https://doi.org/https://doi.org/10.13044/j.sdewes.2015.03
, The development of a thermoelectric power generator dedicated to stove-fireplaces with heat accumulation systems ,Energy Convers Manag , Vol. 125 ,pp 185–193 , 2016, https://doi.org/https://doi.org/10.1016/J.ENCONMAN.2016.05.091
, Design Optimization of Energy Efficient Residential Buildings in Mediterranean Region ,Journal of Sustainable Development of Energy, Water and Environment Systems , Vol. 10 (2),pp 1090385 , 2022, https://doi.org/https://doi.org/10.13044/j.sdewes.d9.0385
, A study of the operation of a wood-fired stove with an incorporated thermoelectric generator , 2021
, Prototypical Biomass-Fired Micro-Cogeneration Systems—Energy and Ecological Analysis ,Energies (Basel) , Vol. 13 (15),pp 3909 , 2020, https://doi.org/https://doi.org/10.3390/en13153909
, Comparative Analysis of Real-Emitted Particulate Matter and PM-Bound Chemicals from Residential and Automotive Sources: A Case Study in Poland ,Energies , Vol. 16 , 2023, https://doi.org/https://doi.org/10.3390/en16186514
, Electrochemical CO2-to-CO conversion: A comprehensive review of recent developments and emerging trends ,Sep Purif Technol , Vol. 330 ,pp 125177 , 2024, https://doi.org/https://doi.org/10.1016/J.SEPPUR.2023.125177
, Polycyclic aromatic hydrocarbons from wood pyrolyis in charcoal production furnaces ,Environ Res , Vol. 101 (3),pp 304–311 , 2006, https://doi.org/https://doi.org/https://doi.org/10.1016/j.envres.2006.01.005
, Nanoparticle emissions from residential wood combustion: A critical literature review, characterization, and recommendations ,Renewable and Sustainable Energy Reviews , Vol. 103 ,pp 515–528 , 2019, https://doi.org/https://doi.org/10.1016/J.RSER.2019.01.007
, Electrical performance analysis and economic evaluation of combined biomass cook stove thermoelectric (BITE) generator ,Bioresour Technol , Vol. 98 (8),pp 1670–1674 , 2007, https://doi.org/https://doi.org/10.1016/j.biortech.2006.05.048
, The design, development and performance evaluation of thermoelectric generator (TEG) integrated forced draft biomass cookstove ,Procedia Computer Science , Vol. 52 (1),pp 723–729 , 2015, https://doi.org/https://doi.org/10.1016/j.procs.2015.05.085
, Study of a TE (thermoelectric) generator incorporated in a multifunction wood stove ,Energy , Vol. 36 (3),pp 1518–1526 , 2011, https://doi.org/https://doi.org/10.1016/J.ENERGY.2011.01.012
, Compact Water-Cooled Thermoelectric Generator (TEG) Based on a Portable Gas Stove ,Energies 2018 , Vol. 1111 (9),pp 2231 ,pp 2231 , 2018, https://doi.org/https://doi.org/10.3390/EN11092231
, A Combined Heat and Power System for Solid-fuel Stoves Using Thermoelectric Generators ,Energy Procedia , Vol. 75 ,pp 597–602 , 2015, https://doi.org/https://doi.org/10.1016/j.egypro.2015.07.462
, Field trial testing of an electricity-producing portable biomass cooking stove in rural Malawi ,Energy for Sustainable Development , Vol. 20 (1),pp 1–10 , 2014, https://doi.org/https://doi.org/10.1016/J.ESD.2014.01.009
, Thermoelectricity - A promising complementarity with efficient stoves in off-grid-areas ,Journal of Sustainable Development of Energy, Water and Environment Systems , Vol. 3 (3),pp 256–268 , 2015, https://doi.org/https://doi.org/10.13044/J.SDEWES.2015.03.0020
,